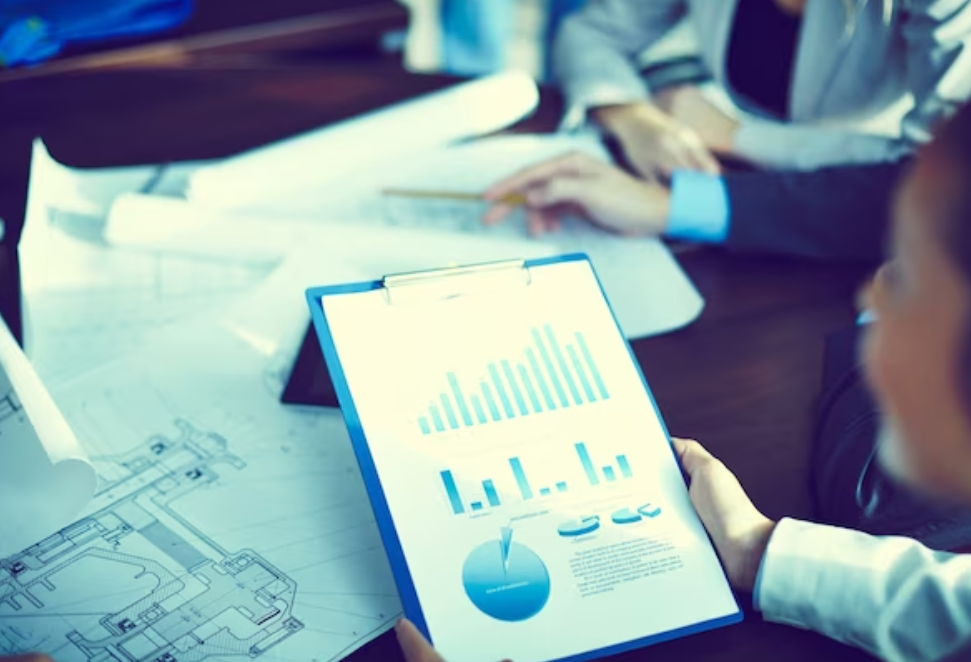
Articles
CO2 capture, utilization and storage (CCUS) is recognized as a uniquely important option in global efforts to control anthropogenic greenhouse-gas (GHG) emissions. Despite significant progress globally in advancing the maturity of the various component technologies and their assembly into full-chain demonstrations, a gap remains on the path to widespread deployment in many countries. In this paper, we focus on the importance of business models adapted to the unique technical features and sociopolitical drivers in different regions as a necessary component of commercial scale-up and how lessons might be shared across borders. We identify three archetypes for CCUS development—resource recovery, green growth and low-carbon grids—each with different near-term issues that, if addressed, will enhance the prospect of successful commercial deployment. These archetypes provide a framing mechanism that can help to translate experience in one region or context to other locations by clarifying the most important technical issues and policy requirements. Going forward, the archetype framework also provides guidance on how different regions can converge on the most effective use of CCUS as part of global deep-decarbonization efforts over the long term.
Article Large-Scale Affordable CO2 Capture Is Possible by 2030 In this study, using a granular analysis of the existing power plant fleet at Shenhua Group, a large power company, we show that local variations in power plant type, operation, geographical location, age, and fuel costs result in significant distribution in the avoided cost of CO2 capture within a single fleet. The fleet analysis initiated in this study is required to estimate the distribution of CO2 capture costs. The strategy addresses the concerns that currently CCS is universally expensive
The cost of delivered H2 using the liquid-distribution pathway will approach $4.3–8.0/kg in the USA and 26–52 RMB/kg in China by around 2030, assuming large-scale adoption. Historically, hydrogen as an industrial gas and a chemical feedstock has enjoyed a long and successful history. However, it has been slow to take off as an energy carrier for transportation, despite its benefits in energy diversity, security and environmental stewardship. A key reason for this lack of progress is that the cost is currently too high to displace petroleum-based fuels. This paper reviews the prospects for hydrogen as an energy carrier for transportation, clarifies the current drivers for cost in the USA and China, and shows the potential for a liquid-hydrogen supply chain to reduce the costs of delivered H2. Technical and economic trade-offs between individual steps in the supply chain (viz. production, transportation, refuelling) are examined and used to show that liquid-H2 (LH2) distribution approaches offer a path to reducing the delivery cost of H2 to the point at which it could be competitive with gasoline and diesel fuel.
Modern society depends on a wide and increasing array of raw materials — from simple steels to esoteric compounds derived from the far corners of the periodic table — to produce the products that enrich our lives and sustain our standard of living. Some of these materials are particularly vulnerable to supply disrup-tions due to natural disasters, geopolitics, or step changes in demand brought about by technological breakthroughs. Materials criticality is concerned with understanding these dynamics and finding ways to ensure adequate near- and long-term supply of these materials.This article introduces methods to identify at-risk, critical materials, and outlines general strategies to address challenges related to these materials. It then highlights some of the technical aspects associated with the produc-tion, processing, and recycling of such materials
For hydrogen to play a meaningful
role in a sustainable energy system,
all elements of the value chain must
scale coherently. Advocates support electrolytic (green) hydrogen
or (blue) hydrogen that relies on
methane reformation with carbon
capture and storage; however, efforts to definitively choose how to
deliver this scaling up are premature. For blue hydrogen, methane
emissions must be minimized.
Best in class supply chain management in combination with high
rates of CO2 capture can deliver a
low carbon hydrogen product. In
the case of electrolytic hydrogen,
the carbon intensity of power
needs to be very low for this to be
a viable alternative to blue
hydrogen. Until the electricity grid
is deeply decarbonized, there is
an opportunity cost associated
with using renewable energy to
produce hydrogen, as opposed
to integrating this with the power
system. To have a realistic chance
of success, net zero transition pathways need to be formulated in a
way that is coherent with socio-political-economic constraints.
Transport electrification is a key element of decarbonization strategies; thus, the design, production, manufacture, use, and disposal of lithium-ion batteries (LIBs) are taking center stage. The environmental, economic, and social consequences of the battery life cycle are high on political agendas, owing to exponential growth in metals extraction; the climate impacts of battery production; and uncertainties in battery end-of-life (EOL) safety, recyclability, and environmental consequences (1) [see figs. S1 to S3 in the supplementary materials]. The European Union (EU) has proposed a new Battery Regulation (2) that intends to ensure sustainability for batteries placed on the EU market (see the figure), developing a robust European battery industry and value chain. The Regulation is very much needed, but, as discussed below, it will have global implications, with perhaps some unintended consequences. If left unaddressed, the Regulation, at worst, could hamper climate change mitigation targets and fall short of its intentions to promote a circular economy and establish a socially acceptable raw material supply chain.
Light emitting diode (LED) lamps use significantly less rare earth elements per lumen of light generated than their fluorescent lamp counterparts. As a result, the global demand for rare earth oxides used in lighting will eventually peak and decline, as the market share of LED lighting increases. The exact timing of this inflection point is dependent on a number of factors, including market growth rates, regional LED adoption rates, and the relative costs of LED and fluorescent lighting technology. Lighting market forecasts from the U.S. Department of Energy were used to estimate the timing of the inflection point for the United States. Domestic rare earth demand can peak as early as 2019. A similar dynamic is expected globally, with timing sensitive to the local LED adoption rate. The analysis did not account for the potential effects of new technologies that use rare earth elements or possible variations in growth rates due to the economic cycle.
Over the past decade, raw material price spikes have called attention to the supply security of a variety of critical materials, including rhenium, rare earth elements, and helium. While market forces play an important role in creating and resolving these situations, transitions in technology also create step-changes in demand that increase or decrease the criticality of different materials. With an appropriate understanding of how materials are used in various applications, it is possible to explore the critical materials implications associated with the introduction of new technologies. Work is already underway to investigate and mitigate the materials impacts of emerging clean energy technologies related to solar power and energy storage. Rapid technological change is also being enabled by information technologies and the Internet of Things (IOT). Here, less work has been done around materials trends and their implications. This paper presents a case study around emerging technologies for data storage and what their implementation at mass scale (zettabyte, ZB) might mean for existing supply chains and market dynamics for certain critical materials.